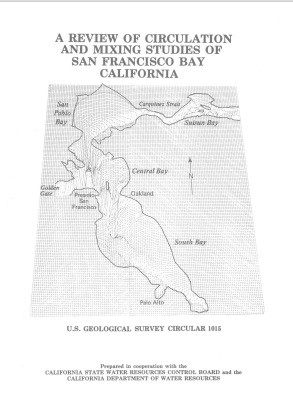
Product Details
- Product Number
- 14920
- Series
- CIR-1015
- Scale
- NO SCALE
- Alternate ID
- 21-1015
- ISBN
- 978-0-607-72588-9
- Authors
- SMITH, LAWRENCE H.
- Version Date
- 01/01/1987
- Media
- Paper
- Format
- Flat
- Digital Reproduction
- Yes
Additional Details
- Description
-
A review of circulation and mixing studies of San Francisco Bay, California
Abstract
A description of the major characteristics and remaining unknowns of circulation and mixing in San Francisco Bay has been constructed from a review of published studies. From a broad perspective San Francisco Bay is an ocean-river mixing zone with a seaward flow equal to the sum of the river inflows less evaporation. Understanding of circulation and mixing within the bay requires quantification of freshwater inflows and ocean-bay exchanges, characterization of source-water variations, and separation of the within-bay components of circulation and mixing processes. Description of net circulation and mixing over a few days to a few months illustrates best the interactions of major components. Quantification of tidal circulation and mixing is also necessary because net circulation and mixing contain a large tide-induced component, and because tidal variations are dominant in measurements of stage, currents, and salinity. The discharge of the Sacramento-San Joaquin Delta into Suisun Bay is approximately 90 percent of the freshwater inflow to San Francisco Bay. Annual delta discharge is characterized by a winter season of high runoff and a summer season of low runoff. For the period 1956 to 1985 the mean of monthly discharges exceeded 1,000 cubic meters per second (35,000 cubic feet per second) for the months of December through April, whereas for July through October, it was less than 400 cubic meters per second (14,000 cubic feet per second). The months of November, May, and June commonly were transition months between these seasons. Large year-to-year deviations from this annual pattern have occurred frequently. Much less is known about the ocean-bay exchange process. Net exchanges depend on net seaward flow in the bay, tidal amplitude, and longshore coastal currents, but exchanges have not yet been measured successfully. Source-water variations are ignored by limiting discussion of mixing to salinity. The bay is composed of a northern reach, which is strongly influenced by delta discharge, and South Bay, a tributary estuary which responds to conditions in Central Bay. In the northern reach net circulation is characterized by the river-induced seaward, flow and a resulting gravitational circulation in the channels, and by a tide- and wind-induced net horizontal circulation. A surface layer of relatively fresh water in Central Bay generated by high delta discharges can induce gravitational circulation in South Bay. During low delta discharges South Bay has nearly the same salinity as Central Bay and is characterized by tide- and wind-induced net horizontal circulation. Several factors control the patterns of circulation and mixing in San Francisco Bay. Viewing circulation and mixing over different time-periods and at different geographic scales causes the influences of different factors to be emphasized. The exchange between the bay and coastal ocean and freshwater inflows determine the year-to-year behavior of San Francisco Bay as a freshwater-saltwater mixing zone. Within the bay, exchanges between the embayments control variations over a season. Circulation and mixing patterns within the embayments and the magnitude of river-induced seaward flow influence the between-bay exchanges. The within-bay patterns are in turn determined by tides, winds, and freshwater inflows. Because freshwater inflow is the only factor that can be managed, a major study focus is estimation of inflow-related effects. Most questions relate to the patterns of freshwater inflow necessary to protect valuable resources whose welfare is dependent on conditions in the bay. Among the important questions being addressed are: --What quantity of freshwater inflow is necessary to prevent salt intrusion into the Sacramento-San Joaquin Delta, and what salinity distributions in the bay would result from various inflow patterns? --What quantity of freshwater inflow is sufficient to flush pollutants through the bay?
- Two Sided
- No
- Pieces
- 1
- Languages
- English